Additional LPCN tests (Fig. 5) were conducted at higher temperatures to investigate cycle time savings. Typical carburizing temperatures of 1,700°F (930°C) and 1,760°F (960°C) were selected for study. Process parameters were similar to the trials at 1,615°F (880°C). GDOS profiles were conducted to determine the nitrogen content at the near surface up to 0.0012" (30 ìm). Results indicated a nitrogen content of 0.5%, falling rapidly to 0.15% below the near surface at 1,700°F (930°C) and the same behavior was observed at 1760°F (960°C). The nitrogen profile was achieved at 1,700°F (930°C) up to 0.015" (0.4 mm) and up to 0.40" (1.0 mm) at 1,760°F (960°C). These tests indicate that the process can work at high temperatures as well.
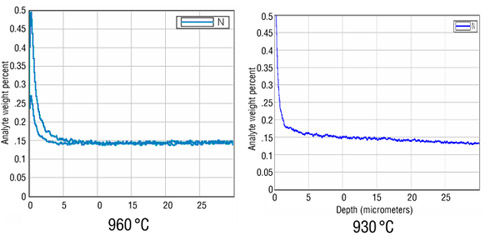
Figure 5—LPCN elevated temperature test results.
Fatigue and Impact Studies
Specification targets (Table 2) were selected for gears (Fig. 6) of SAE 5130 (29MnCr5) material.
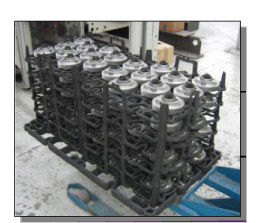
Figure 6—Gear test load.
Hardness and effective case depth results (Table 3) achieved targeted values. This confirmed that there was no metallurgical difference to influence fatigue strength results.
One of the mechanical property tests employed was to determine impact properties. Impact samples (Fig. 7) were tested in a pendulum type impact tester at 50J.
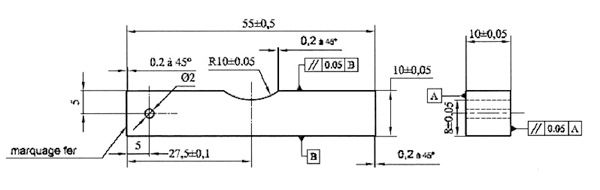
Figure 7—Impact sample.
The result of impact testing when compared to LPC + HPGQ revealed, as one might expect, a strong benefit of tempering on impact properties. Noteworthy is the improvement over LPC + HPGQ achieved by interrupted quenching or by LPCN + HPGQ. These results indicate that additional testing is required to optimize results. The use of LPCN + HPGQ with StopGQ quenching (Fig. 8) resulted in impact values exceeding those of LPC + HPGQ + tempering.
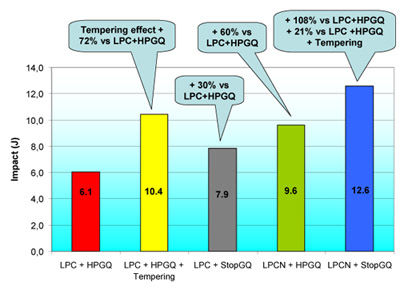
Figure 8—Improvement of impact properties over LPC+HPGQ by process.
Another mechanical test employed was that of rotating bending fatigue involving a notched sample geometry (Fig. 9). The test was run for 1 x 107 cycles.
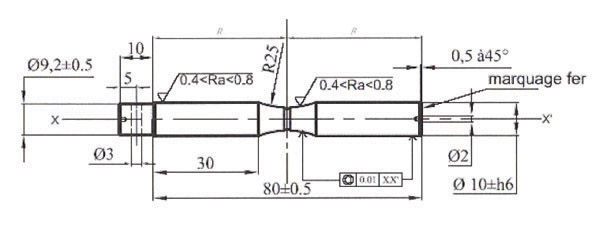
Figure 9—Rotating bending fatigue sample.
The results of rotating bending fatigue testing (Fig. 10) indicate all of the new processes improve strength values over those of either LPC + HPGQ or LPC + HPGQ + tempering.
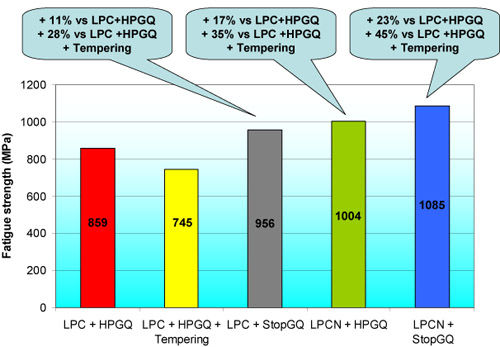
Figure 10—Improvement of rotating beam fatigue properties over LPC+HPGQ by process.
Realized Objectives
An improvement in fatigue strength was realized using the “auto-tempering” effect achieved by StopGQ quenching. The effect of nitrogen present in the surface layer of LPCN parts was revealed in higher impact and fatigue strength values (Fig. 11).
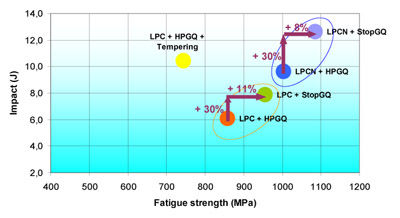
Figure 11a—Summary of StopGQ® benefits versus LPC+HPGQ.
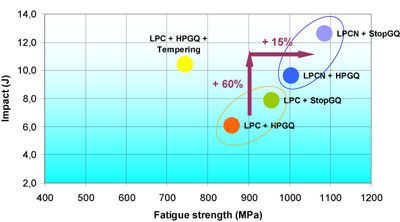
Figure 11b—Summary of LPCN benefits versus LPC+HPGQ.
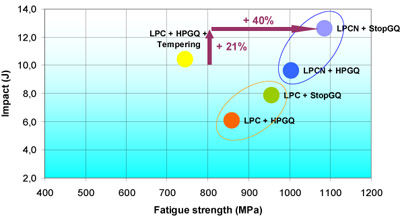
Figure 11c—Summary of LPCN benefits versus LPC+HPGQ + tempering.
Dilatometry studies (Fig. 12) showed less contraction with an interrupted quench compared to direct high-pressure gas quenching. During the StopGQ quench, quadratic martensite is transformed into cubic martensite plus å carbides, resulting in an automatic tempering effect. This was demonstrated by rupture analysis (Fig. 13), which revealed higher ductility in the core of the material processed with LPCN + StopGQ quenching.
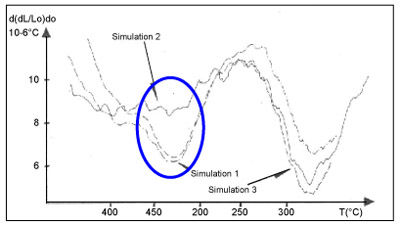
Figure 12—Dilatometry studies of the StopGQ quenching method.
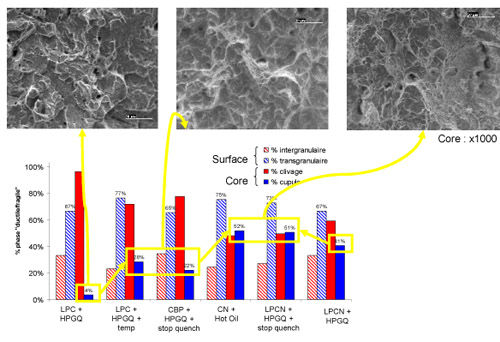
Figure 13—Rupture analysis comparison by process.
Scanning electron microscopy (SEM) analysis at 3,700X comparing LPC and LPCN microstructures found fine precipitates of carbonitrides in the latter, which are believed to have a strong influence on fatigue strength (Fig. 14).
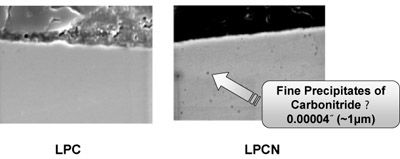
Figure 14—Comparison of LPC and LPCN microstructures.
These trials allowed the following conclusions to be reached:
• Conditions were established to
predict and control carbon and nitrogen concentration profiles.
• The metallurgical parameters which act on resistance in fatigue inflec- tion of gear teeth for a fixed
hardened depth were better under- stood.
• Results indicate improvements in fatigue resistance of gear teeth
compared to low-pressure vacuum
carburizing treatments applied to gear boxes.
Future Studies
Future research and development efforts will target further understanding of the role of nitrogen on mechanical properties. Those efforts will include microstructural analysis of grain boundaries to determine if the grain size has been refined. In addition, further optimization of the influence of StopGQ quenching temperature and hold time will be investigated.
Acknowledgment
The author would like to thank Ascometal for their assistance in the testing and evaluation of certain technical results presented in this article.
Aymeric Goldsteinas leads the Prospective and Innovation team at ECM in Grenoble, France. Acting as liaison between R&D and sales, he demonstrates ECM’s technologies to customers worldwide. He began with ECM in 1998 as an R&D engineer and helped to create the patented Infracarb process with acetylene. He has also helped develop and patent several other heat treating processes, including low pressure vacuum carburizing and high pressure gas quenching processes. Goldsteinas holds a bachelor’s degree in life sciences and a master’s degree in science and technology.